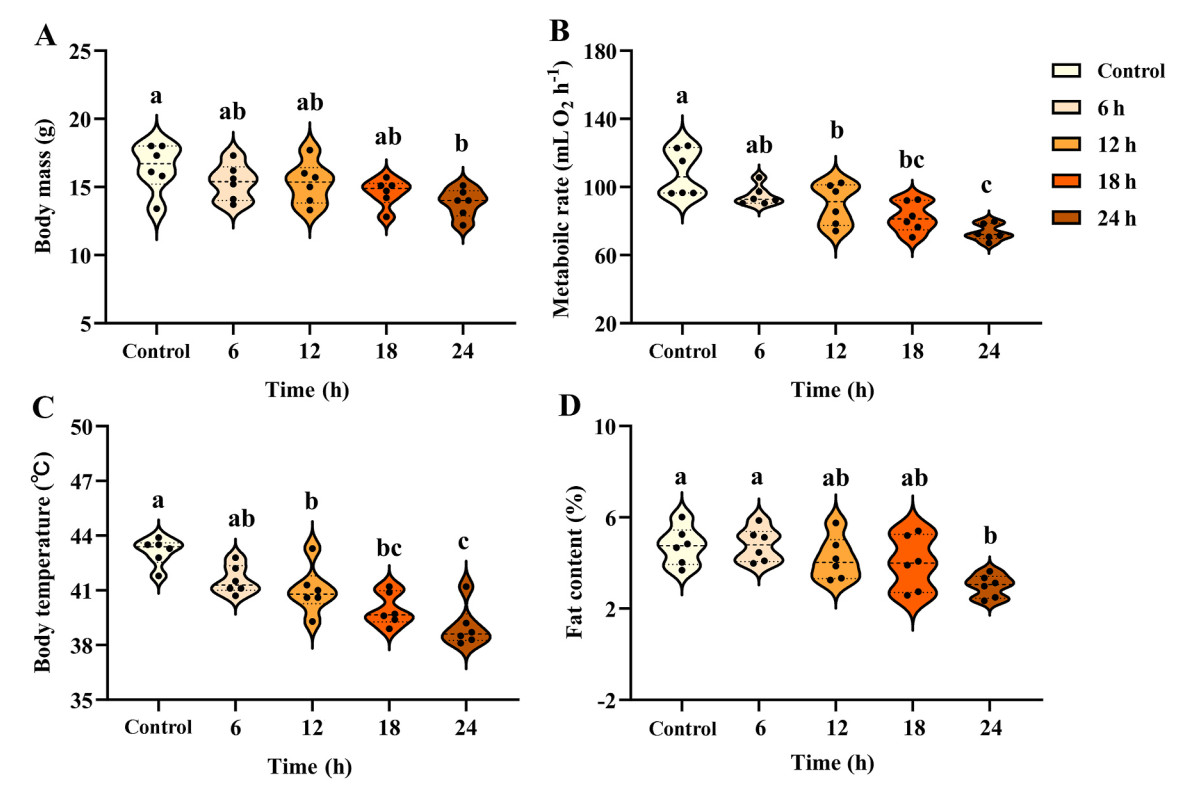
Citation: | Hong Jin, Qi Xia, Ziqing Gao, Yujie XuanYuan, Beibei Dong, Ming Li, Qingjian Liang, Jinsong Liu. 2025: Decreased basal thermogenesis is associated with the downregulation of cellular metabolic activity of organs and serum thyroid hormones in fasting Eurasian Tree Sparrows (Passer montanus). Avian Research, 16(1): 100220. DOI: 10.1016/j.avrs.2024.100220 |
Food is a critical environmental factor that influences animal survival, especially for small passerines due to their high mass-specific metabolic rates. Basal metabolic rate (BMR) reflects the energy expended by endothermic animals for basic physiological processes and constitutes a major part of their daily energy budget. Some birds have been shown to employ compensatory mechanisms during food shortages, temporarily reducing these self-maintenance expenditures without using hypothermia. However, the mechanisms of BMR adjustment remain unexplored. In the present study, we assessed the phenotypic variation in basal thermogenesis of Eurasian Tree Sparrows (Passer montanus) by comparing a control group to groups fasted for 6, 12, 18, and 24 h. We focused on the correlation between a reduction in energy metabolism and the alterations of cellular metabolic activities, mitochondrial substrate supply, and changes in serum thyroid hormones during fasting. Our data indicated that fasting groups had significantly lower body mass, BMR, body temperature, and body fat content. Furthermore, fasting groups had significantly lower glycogen levels, mitochondrial state 4 respiration and cytochrome c oxidase (CCO) activity in the liver, and CCO activity in pectoral muscle. The levels of avian uncoupling protein (av-UCP) mRNA were significantly reduced, while the levels of myostatin protein in pectoral muscle were significantly increased in the fasting groups. Furthermore, the groups subjected to fasting exhibited significantly lower levels of serum glucose, triglyceride, thyroxine (T4), and triiodothyronine (T3). Positive correlations were observed between the following pairs of variables: log BMR and log body mass, log body mass and log body fat, log BMR and log state 4 respiration in the liver, log BMR and log CCO activity in the liver and muscle, log BMR and log av-UCP mRNA expression, whereas a negative correlation was observed between log BMR and log myostatin level. In addition, a positive correlation was also detected between log T3 and each of the following: log BMR, state 4 respiration, and log CCO activity in the liver. Our results suggested that decreased metabolic thermogenesis via down-regulation in cellular aerobic capacity of organs and serum thyroid hormones may be an important survival strategy for fasting Tree Sparrows to reduce energy expenditure.
Food serves as a crucial environmental determinant that impacts animal survival (Foster, 1997; Gutiérrez et al., 2011). The life cycles of numerous animal species encompass prolonged durations of food scarcity, which have been shown to be particularly challenging to the animals (Foster, 1997; Hohtola, 2012). Compared to mammals, birds are particularly vulnerable to these inevitable food shortages because of the elevated metabolic heat production required for maintaining high body temperatures of 40–42 ℃ and the energy demand of flight (Prinzinger et al., 1991; Hohtola, 2012; Xu et al., 2024).
Food shortage is common during the life history of small birds, and it may happen as a result of seasonal changes in food quantity and quality or short-term food restrictions facing migrating birds during long flight periods (Karasov et al., 2004). In the face of unpredictable food shortages, birds have evolved various morphological, physiological, and behavioral adaptations to enhance their survival during periods of starvation (Hiebert, 1991; Pierce and McWilliams, 2004). It has been shown that food availability influences various physiological parameters, including the body mass (Secor and Carey, 2016), body composition (Kelly and Weathers, 2002; Zhang et al., 2018a), and basal thermogenesis (Gutiérrez et al., 2011; Liang et al., 2015; Xu et al., 2024). Changes in body mass typically reflect alterations in the mass of various tissues and organs. For example, Chinese Bulbuls (Pycnonotus sinensis) were found to have a lighter heart, liver, spleen, and digestive tract in response to food restriction (Liang et al., 2015; Zhang et al., 2018a). Generally, different tissues and organs experience varying degrees of mass loss during fasting (Secor and Carey, 2016; Xu et al., 2024). Most of the mass lost during food restriction is attributable to body fat reduction. Studies have indicated that food-restricted animals have decreased body mass and approximately 50% less fat mass compared with those that were given ad libitum feeding (Liang et al., 2015; Zhang et al., 2018a), highlighting the impact of food deprivation on avian body composition (Mao et al., 2019; Xu et al., 2024).
Basal metabolic rate (BMR) represents the minimum energy required for a non-reproductive adult animal at rest, in a post-absorptive state, and within the thermoneutral zone (McNab, 1997, 2009; Swanson et al., 2017). BMR is a critical physiological parameter for evaluating the energy costs associated with thermogenesis (Zheng et al., 2008a; Zhou et al., 2016; Li et al., 2022; Swanson et al., 2017). Birds that are subjected to food restriction or fasting often exhibit reduced BMR compared with those given ad libitum feeding, although this has not been universally observed (Liang et al., 2015; Mao et al., 2019; Xu et al., 2024). This reduction has been documented across various species, including Thrush Nightingales (Luscinia luscinia; Klaassen et al., 1997), White Storks (Ciconia ciconia; Mata et al., 2001), Blackcaps (Sylvia atricapilla; Karasov et al., 2004), and Silky Starlings (Spodiopsar sericeus; Mao et al., 2019). BMR in small birds is a flexible trait modulated at multiple organizational levels (Swanson, 2010; Zheng et al., 2014a; Li et al., 2024). At the organ level, changes in the size or mass of metabolically active tissues and organs (e.g., liver, heart, lung, kidneys, gizzard and small intestine) can significantly contribute to avian BMR variation (Daan et al., 1990; Liu and Li, 2006; Clapham, 2012; Li et al., 2017, 2024). At the cellular level, adjustments in physiological and biochemical regulations might also influence BMR in birds (Zhou et al., 2016; Li et al., 2024). Changes in catabolic enzyme activity and/or the capacity for metabolic substrate supply can influence the metabolic activity of organs, thereby affecting metabolic rates at the organismal level (Swanson, 1991; Zheng et al., 2008b, 2014a). Mitochondrial respiration generates heat from its imperfect coupling to ADP phosphorylation, with state 4 respiration (an index of resting mitochondrial respiration rates) serving as an indicator for tracking BMR changes (Zheng et al., 2013; Zhou et al., 2016; Li et al., 2024). Enhanced catabolic enzyme activity in oxidative pathways can also improve cellular aerobic capacity and, therefore, increase heat production (Liknes and Swanson, 2011; Zhou et al., 2016; Li et al., 2022). Changes in cytochrome c oxidase (CCO, complex Ⅳ of the respiratory chain) activity serve as a common enzymatic indicator of altered BMR at the cellular level (Zheng et al., 2008b, 2014a; Li et al., 2022). Furthermore, adjustment of substrate metabolism might also contribute to variation in BMR in birds (Swanson, 1991; Xu et al., 2024). For example, birds and mammals tend to deplete their glycogen reserves during the early stages of fasting (Didier et al., 1983; Parilla, 1978). Lipids can serve as an effective metabolic fuel during fasting because of their high energy density (about 39 kJ g−1) and significant dehydration during storage (Allen, 1976). During fasting, the products derived from the hydrolysis of triglycerides can be used to produce glucose via gluconeogenesis to maintain blood glucose homeostasis (Totzke et al., 1999). On the other hand, fatty acids, the hydrolytic products of lipids, can be transported into the mitochondria for β-oxidation, so fatty acids can provide substrates for cellular metabolism (Stager et al., 2015; Xu et al., 2022). Skeletal muscle, which constitutes nearly 40% of body mass, is also a primary thermogenesis contributor (Weber and Piersma, 1996; Zheng et al., 2008b; Galt et al., 2014; Zhou et al., 2016; Li et al., 2022). In birds, avian uncoupling protein (av-UCP) mediates the uncoupling of oxidative phosphorylation and thermogenesis in skeletal muscle (Toyomizu et al., 2002; Ueda et al., 2005), making analysis of the av-UCP expression relevant to the assessment of the production of reactive oxygen species and thermogenesis (Toyomizu et al., 2002; Ouchi et al., 2021). A decrease in av-UCP expression may indicate reduced energy metabolism in fasting birds (Abe et al., 2006; Zhang et al., 2018a; Xu et al., 2024). Furthermore, the regulation of skeletal muscle mass involves myostatin, an autocrine/paracrine inhibitor of muscle growth belonging to the TGFβ family (Amthor et al., 2002; Zhang et al., 2018b; Xu et al., 2024). Studies have established a correlation between fluctuation in muscle mass and alteration in myostatin expression that occurs among avian species during migratory periods or the process of winter acclimatization (Swanson et al., 2009, 2014; Price et al., 2011).
In addition to these morphological and physiological adjustments, the metabolism of endotherms may also be affected by thyroid hormones (Yen, 2001; Silva, 2006; Zheng et al., 2008b, 2014a; Tang et al., 2022). These hormones (thyroxine T4 and triiodothyronine T3) are essential for central body temperature regulation, thermogenesis stimulation, and cellular metabolism control (Silva, 2006; Wen et al., 2019; Xu et al., 2024). The absence of thyroid hormones can reduce basal energy expenditure by at least 30% (Enrique and Silva, 2003; Liu et al., 2006; Zheng et al., 2013). Decreased serum levels of thyroid hormones have been detected in birds undergone long-term fasting, and these include Emperor Penguins (Aptenodytes forsteri; Groscolas, 1986), chickens (Okumura and Kita, 1999), and Japanese Quails (Coturnix japonica; Xu et al., 2024). However, this phenomenon requires further confirmation because of the limited reports on thyroid hormone level changes in small birds, such as Eurasian Tree Sparrows (Passer montanus) under fasting conditions.
The Eurasian Tree Sparrow, a small granivorous bird, is widely distributed across Europe and Asia (MacKinnon and Phillipps, 2000). This species was chosen for our study because it is a common passerine in China, with previous research providing essential background information (Liu and Li, 2006; Liu et al., 2008; Zheng et al., 2008b, 2014b; Li et al., 2020; Tang et al., 2022). During the breeding season, Eurasian Tree Sparrows primarily feed on arthropods (insects and spiders), while in autumn and winter, they switch to fruits and seeds (Zheng et al., 2008b). This dietary shift exposes them to significant fluctuations in food quality and availability, making it relevant to study the adaptations that support this flexibility. Previous research has focused on how food deprivation leads to significant decreases in the mass of body fat, metabolically active organs (e.g., liver, kidney, and digestive tract), and catabolic enzyme activities (state 4 respiration and CCO activity) in Chinese Bulbuls and Silky Starlings (Liang et al., 2015; Zhang et al., 2018a, 2018b; Mao et al., 2019). We also found that long-term fasting affects mainly the lipid metabolism of female adult Japanese Quails (Xu et al., 2024). However, the mechanisms underlying basal thermogenesis adjustments that allow Tree Sparrows to adapt to fasting remain unknown. This study aimed to reveal the multi-level adaptive regulations involved in basal thermogenesis of Tree Sparrows during fasting using an integrative biology approach. We hypothesized that Tree Sparrows would show a low level of metabolic thermogenesis during periods of food restriction, a result of coping with food shortages. We measured various thermogenic indicators in both the control and fasting groups of birds. These indicators included BMR, glycogen levels, mitochondrial state 4 respiration, and CCO activity in the liver and pectoral muscle, and av-UCP mRNA and myostatin protein levels in the pectoral muscle, as well as serum glucose, triglyceride, T4, and T3 levels. We predicted the sparrows would show lower levels of BMR, state 4 respiration, and CCO activity and decreased T3 and T4 concentrations in response to food restriction. Moreover, Tree Sparrows would down-regulate the expression of the av-UCP gene and up-regulate the expression of myostatin. These findings could shed light on the thermogenic adaptations in small granivorous birds that help them to cope with food scarcity.
This study was conducted in Wenzhou City, Zhejiang Province (27°29ʹ N, 120°51ʹ E, 14 m above sea level), China. Wenzhou is characterized by a warm-temperate climate with a mean annual precipitation of 1500 mm and a daily temperature ranging from 28 to 39 ℃ in July to 3–8 ℃ in January (Zheng et al., 2014a; Li et al., 2024).
During October in 2017, adult Eurasian Tree Sparrows (individual sex not determined) were captured by mist net from a wild population in Wenzhou. After capture, the birds were transported to Wenzhou University, where they were individually weighed and housed in 50 cm × 30 cm × 30 cm stainless-steel cages at room temperature (25 ± 1 ℃) under a 12:12-h light-dark cycle with lights on at 6:00 a.m. While in captivity, the birds were provided with ad libitum access to food, which included seeds (millet and sunflower seed), protein supplement (consisting of a homogenized mixture of commercial pellets with a minimum protein content of 20%, and hard-boiled eggs), and water for 2 weeks. Subsequently, individuals were randomly divided into five groups, with each group consisting of six animals (n = 6). These were the control group (n = 6), which had continuous access to food and water, and four fasting groups, each with a different duration of fasting (6, 12, 18, and 24 h), all of which were only supplied with water (Zhang et al., 2018a).
Metabolic rates of the Tree Sparrows were examined with an open-circuit respirometry system based on the S-3A/I oxygen analyzer (AEI Technologies, Pittsburgh, PA). The birds were individually placed in separate 1.5-L plastic metabolism chambers (custom-made and contained a perch for the bird to stand on) within a temperature-controlled cabinet (Artificial Climatic Engine BIC-300; Boxun, Shanghai, China) capable of regulating the temperature to within 0.5 ℃ of a defined value. The set temperature was 25 ± 0.5 ℃, which was within the thermal neutral zone for Eurasian Tree Sparrows (Zheng et al., 2008b). Airflow was pulled through the chamber maintained at 300 mL min−1 using a flow control system (AEI Technologies R-1, USA) calibrated to ±1% accuracy with a digital bubble-meter (TSI 4100 Series, USA), with CO2 and water vapor removed by Ascarite and Drierite, respectively (Tang et al., 2022). Oxygen concentration was determined using an oxygen sensor (AEI Technologies N-22M) at 10-s intervals. Baseline O2 concentrations were measured about 1 h and obtained before and after each test (one in the evening and one in the morning; Hu et al., 2017; Tang et al., 2022). BMR was measured for all birds for a period of at least 1 h following 1 h equilibration periods (Zheng et al., 2008b, 2014a). BMR was calculated for each individual as the average of the 30 lowest consecutive oxygen consumption recordings (about 5 min) (Zheng et al., 2014a). Metabolic rates of sparrows were calculated using Equation (2) derived by Hill (1972). The readings were converted to mL O₂ h−1 and corrected for standard temperature, pressure, and dry gas conditions (Schmidt-Nielsen, 1997). Food was removed 4 h before each test to provide a postabsorptive condition. Measurements were taken during the rest phase (2000-0400) in dark chambers. The mean body mass of each experimental group was calculated by measuring the body mass of each bird before and after the experiment. Body mass was measured using an electronic balance (Sartorius BT2S, Germany) with an accuracy of 0.1 g. Body temperature was taken before BMR measurements using a lubricated thermocouple inserted into the cloaca of the bird to a depth of 1–2 cm, and the output was digitized using an Oakton thermocouple meter (Eutech Instruments, Singapore).
After metabolic measurements were completed, each bird was decapitated, and the blood was collected from the jugular vein into a 1.5-mL EP tube for serum preparation. Organs were excised and weighed to the nearest 0.1 mg on an electronic balance (Sartorius BSA124S-CW, Germany). Tissue samples from the liver and pectoral muscle were snap-frozen in liquid nitrogen and stored at −80 ℃ for further analyses. The remaining carcass was dried at 65 ℃ to determine its dry mass, and total body fat was extracted using a Soxhlet apparatus (Foss Instrument, Germany). Fat content was calculated as a percentage of the wet carcass mass (Wu et al., 2014).
State 4 respiration was measured using a DW1/AD chamber with an integral Clark-type polarographic oxygen electrode (Hansatech Instruments LTD, England) as previously described (Li et al., 2024) at 30 ℃ and over a 1-h cycle. The same electrode was used to polarographically measure the activities of CCO activity at 30 ℃ as in previous studies (Bai et al., 2016; XuanYuan et al., 2023). Both parameters were recorded as nmol O2 min−1 organ−1 (whole tissue−1).
Total RNA from the sparrow pectoral muscle was isolated using an E.Z.N.A. Total RNA Extraction Kit (R6834-02, Omega) and then used as a template to make the cDNA samples by utilizing a Reverse Transcription System (A3500, Promega). The cDNA samples obtained were used to determine the level of av-UCP mRNA via qRT-PCR. The mRNA level of av-UCP was analyzed using a Light Cycler 480 Detection System (Roche, Switzerland). The av-UCP primers used for the amplification were 5ʹ-CGGTGGATGTGGTGAAGACG-3ʹ (forward) and 5ʹ-AGGAAGGASGGGACGACGAAGC-3ʹ (reverse) designed according to the cDNA sequence of Chinese bulbul and chickens (Rey et al., 2008; Mao et al., 2019).
Myostatin expression in the muscle was analyzed by Western blot. The muscle tissue was first homogenized on ice in a buffer containing 50 mM Tris/HCl, pH 7.4, 150 mM NaCl, 1 mM EDTA, 1% SDS, 0.1% Deoxycholate, 1% NP-40, and 1 mM PMSF. The protein concentration in the extract was then determined using an improved protein assay, and 20 μg of protein was resolved by SDS-PAGE using a 12% gel. After electrophoresis, the proteins in the gel were transferred to a nitrocellulose membrane and analyzed by Western blot analysis as described by Zhang et al. (2015). Goat-anti-myostatin antibody (R & D Systems, USA) was used as the primary antibody and anti-goat IgG antibody conjugated to horseradish peroxidase as the secondary antibody (Sangon Biotech, China). The blot was visualized with a chemiluminescent detection ECL reagent (Sangon Biotech, China), followed by exposure to Vilber Lourmat (BitTek Instruments, Inc., USA). The intensity of the band that corresponded to myostatin was quantified using Image-Pro Plus (Media Cybernetics, USA).
Blood samples were obtained and allowed to clot spontaneously at ambient temperature. The sample was centrifuged at 1000×g and 4 ℃ for 10 min. The serum obtained was then used for direct analysis or transferred in clean sample tubes and kept at −20 ℃ for further treatments. Serum glucose (Rongsheng Pharmaceutical Co., China) and triglyceride (Jianchen Pharmaceutical Co., China) levels were determined using a colorimetric commercial kit adapted to microplates. The glycogen concentration in both the liver and pectoral muscle was determined using a coupled enzyme assay following the methodology outlined in the Glycogen Assay Kit manual (Sigma, USA). T4 and T3 concentrations in the serum were analyzed by an ELISA kit (USCN, USA) using a 96-well microplate spectrophotometer (Spectra Max 450; BioTek, USA).
Data were analyzed using SPSS 20.0, with results expressed as mean ± SE. Normality was tested using the Shapiro–Wilk test. The effects of fasting were examined using one-way ANOVA. These included the effects on body mass, organ/tissue masses (except the pectoral muscle), serum glucose, triglyceride, T4, and T3 levels, muscle glycogen, state 4 respiration, and CCO activity in the liver and pectoral muscle, as well as av-UCP and myostatin expression in the pectoral muscle. The effects of fasting on the pectoral muscle mass and hepatic glycogen were analyzed using the Kruskal–Wallis test because the data was not normally distributed. To analyze the effect of fasting on metabolic rate, one-way ANCOVA with body mass as the covariate was used. Body fat content was expressed as a percentage. However, as the body fat content is not typically normally distributed, an arcsine-square root transformation was performed before carrying out the statistical analysis. The Tukey’s post hoc test was used to identify significant differences between groups. The assessment of the relationship between two variables was conducted through least-squares linear regression analysis. Specifically, the association between BMR and organ mass was evaluated utilizing both allometric and residual correlation methods. To mitigate potential statistical complications arising from part-whole correlations, the mass of the organ in question was derived by subtracting the wet organ mass from the total body mass (Christians, 1999). In instances where significant allometric correlations were identified for organ masses, the residuals of the logarithmic transformations of organ masses and BMR were computed based on the allometric equations. These residuals were subsequently subjected to regression analysis to ascertain whether a significant correlation existed between organ masses and BMR. Conversely, in situations where allometric correlations did not reach significance, the untransformed values were regressed against the logarithmic BMR to evaluate potential correlations between BMR and organ mass. A P-value threshold of less than 0.05 was established to denote statistical significance.
In general, birds subjected to fasting exhibited a notable reduction in body mass (F4,25 = 2.973, P = 0.039; Fig. 1A), BMR (F4,25 = 11.354, P < 0.001; Fig. 1B), body temperature (F4,25 = 14.899, P < 0.001; Fig. 1C), and body fat (F4,25 = 4.590, P = 0.006; Fig. 1D) compared with the control ones. Even after adjusting for body mass differences using ANCOVA, BMR still varied significantly among groups (F4,24 = 6.909, P = 0.001). Collectively, the data revealed positive correlations between log body mass and log BMR (r = 0.821, P < 0.001; Fig. 2A) and between log fat content and log body mass (r = 0.448, P = 0.013; Fig. 2B).
Fasting significantly influenced the masses of the liver, heart, lung, spleen, brain, gizzard, small intestine, and total digestive tract (Table 1). However, no significant changes were observed in the masses of the pectoral muscle or kidney (Table 1). Positive allometric relationships between log organ mass and log body mass (excluding organ wet mass) were significant for the pectoral muscle, liver, heart, spleen, small intestine, and total digestive tract (Table 2). The slopes of these allometric regression lines exceeded 1.0 for the pectoral muscle, spleen, and small intestine, indicating a faster rate of decrease in organ masses relative to the overall body mass. Residual correlations showed significant positive associations between BMR residuals and the residuals of liver, lung, spleen, and total digestive tract mass, but not with the residuals of the other organs (Table 2). The residuals pertaining to small intestine mass exhibited a positive correlation with the residuals of BMR; however, this association did not attain statistical significance (P = 0.056, Table 2).
Parameters | 0 h (n = 6) | 6 h (n = 6) | 12 h (n = 6) | 18 h (n = 6) | 24 h (n = 6) | P | |
Wet mass (g) | |||||||
Pectoral muscle | 1.76 ± 0.13 | 1.89 ± 0.12 | 2.06 ± 0.12 | 1.89 ± 0.12 | 2.05 ± 0.13 | χ2 = 2.933 | 0.569 |
Liver | 0.79 ± 0.07 a | 0.80 ± 0.06 a | 0.79 ± 0.06 a | 0.65 ± 0.06 ab | 0.49 ± 0.07 b | F4,24 = 3.650 | 0.018 |
Heart | 0.19 ± 0.01ab | 0.20 ± 0.01a | 0.20 ± 0.01a | 0.17 ± 0.01b | 0.17 ± 0.01b | F4,24 = 2.870 | 0.045 |
Kidney | 0.16 ± 0.02 | 0.18 ± 0.01 | 0.19 ± 0.01 | 0.19 ± 0.01 | 0.16 ± 0.02 | F4,24 = 1.065 | 0.395 |
Lung | 0.16 ± 0.01b | 0.20 ± 0.01a | 0.17 ± 0.01b | 0.16 ± 0.01b | 0.15 ± 0.01b | F4,24 = 3.950 | 0.013 |
Spleen | 0.03 ± 0.00 a | 0.03 ± 0.00 a | 0.02 ± 0.00 ab | 0.02 ± 0.00 bc | 0.01 ± 0.00 c | F4,24 = 4.316 | 0.009 |
Brain | 0.77 ± 0.03a | 0.81 ± 0.03a | 0.69 ± 0.03b | 0.77 ± 0.03a | 0.77 ± 0.03a | F4,24 = 3.173 | 0.032 |
Gizzard | 0.52 ± 0.03ab | 0.47 ± 0.03abc | 0.54 ± 0.03a | 0.46 ± 0.03bc | 0.41 ± 0.03c | F4,24 = 3.186 | 0.031 |
Small intestine | 0.54 ± 0.05a | 0.55 ± 0.04a | 0.51 ± 0.04a | 0.42 ± 0.04b | 0.35 ± 0.05b | F4,24 = 3.061 | 0.036 |
Total digestive tract | 1.10 ± 0.06a | 1.08 ± 0.05a | 1.11 ± 0.05a | 0.91 ± 0.05b | 0.80 ± 0.06b | F4,24 = 5.686 | 0.002 |
Note: Statistical significance was determined by one-way ANCOVA except for pectoral muscle. For pectoral muscle, statistical significance was determined by Kruskal–Wallis. Data are shown as mean ± SE from 30 birds. Different superscript letters in the same row indicate significant differences among groups after Tukey’s post hoc test. P-values in bold indicate differences with statistical significance. |
Allometric correlationsa | Pectoral muscle | Liver | Heart | Kidney | Lung | Spleen | Brain | Gizzard | Small intestine | Total digestive tract | |
r | 0.561 | 0.385 | 0.642 | 0.182 | 0.231 | 0.445 | 0.254 | 0.171 | 0.468 | 0.447 | |
P | 0.001 | 0.035 | <0.001 | 0.337 | 0.221 | 0.014 | 0.175 | 0.366 | 0.009 | 0.013 | |
Slope | 1.012 | 0.979 | 0.855 | 0.325 | 0.333 | 1.988 | 0.223 | 0.237 | 1.389 | 0.767 | |
Residual correlationsb | |||||||||||
r | 0.146 | 0.492 | 0.351 | 0.114 | 0.398 | 0.423 | 0.237 | 0.247 | 0.353 | 0.379 | |
P | 0.442 | 0.006 | 0.057 | 0.550 | 0.029 | 0.020 | 0.208 | 0.188 | 0.056 | 0.039 | |
Slope | −0.094 | 0.202 | 0.332 | 0.091 | 0.398 | 0.102 | 0.389 | 0.258 | 0.130 | 0.238 | |
a Linear regression statistics for log organ mass versus log body mass (minus wet mass of the organ) allometric equations (allometric correlations). b Linear regression statistics for log organ mass residuals versus log BMR residuals (residual correlations), or log organ mass versus log BMR raw values if allometric equations were not significant. P-values in bold indicate differences with statistical significance. |
By the end of the experiment, the fasting groups showed a notable reduction in state 4 respiration within the liver but not in the pectoral muscle (Table 3). The fasting groups also displayed decreased CCO activity in the liver and pectoral muscle (Table 3). Similarly, glycogen levels in liver and pectoral muscle were markedly lower in the fasting birds compared with the control ones (Table 4). Furthermore, positive correlations were identified between log state 4 respiration and log BMR in the liver (r = 0.587, P < 0.001; Fig. 3A), as well as between log CCO activity and log BMR in both the liver (r = 0.598, P < 0.001; Fig. 3B) and pectoral muscle (r = 0.570, P = 0.001; Fig. 3D). However, log state 4 respiration did not correlate with log BMR in the pectoral muscle (r = 0.270, P = 0.149; Fig. 3C).
Variable | Control (n = 6) | 6 h (n = 6) | 12 h (n = 6) | 18 h (n = 6) | 24 h (n = 6) | F4,25 | P |
Liver: | |||||||
State 4 respiration (μmol O2 min−1 organ−1) | 0.27 ± 0.03a | 0.27 ± 0.01a | 0.26 ± 0.03a | 0.25 ± 0.02ab | 0.18 ± 0.01b | 2.846 | 0.045 |
Cytochrome c oxidase activity (μmol O2 min−1 organ−1) | 0.12 ± 0.01a | 0.12 ± 0.01ab | 0.11 ± 0.01ab | 0.10 ± 0.00ab | 0.09 ± 0.01b | 3.966 | 0.013 |
Muscle: | |||||||
State 4 respiration (μmol O2 min−1 whole tissue−1) | 1.01 ± 0.13 | 1.05 ± 0.18 | 0.98 ± 0.08 | 0.91 ± 0.10 | 0.88 ± 0.05 | 0.379 | 0.822 |
Cytochrome c oxidase activity (μmol O2 min−1 whole tissue−1) | 0.49 ± 0.06a | 0.44 ± 0.02a | 0.45 ± 0.04a | 0.41 ± 0.03ab | 0.29 ± 0.03b | 4.398 | 0.008 |
Note: statistical significance was determined by one-way ANOVA. Data are shown as mean ± SE from 30 birds. Different superscript letters in the same row indicate significant differences among groups after Tukey’s post hoc test. P-values in bold indicate differences with statistical significance. |
Parameters | 0 h (n = 6) | 6 h (n = 6) | 12 h (n = 6) | 18 h (n = 6) | 24 h (n = 6) | P | |
Liver glycogen (mg g−1) | 2.66 ± 0.15a | 1.77 ± 0.22a | 1.12 ± 0.07ab | 0.81 ± 0.06b | 0.79 ± 0.04b | χ2 = 25.144 | <0.001 |
Muscle glycogen (mg g−1) | 1.22 ± 0.16a | 0.89 ± 0.07 ab | 0.84 ± 0.08 abc | 0.65 ± 0.09bc | 0.45 ± 0.07c | F4,25 = 7.877 | <0.001 |
Note: For muscle glycogen, statistical significance was determined by one-way ANOVA; for liver glycogen, statistical significance was determined by Kruskal-Wallis. Data are shown as mean ± SE from 30 birds. Different superscript letters in the same row indicate significant differences among groups after Tukey’s post hoc test. P-values in bold indicate differences with statistical significance. |
Birds that were fasted showed a significant decrease in the level of av-UCP mRNA in the pectoral muscle compared with the control birds (F4,25 = 3.790, P = 0.015; Fig. 4A). However, birds in the fasting groups showed a significant increase in the level of myostatin protein in the pectoral muscle compared with the control ones (F4,25 = 6.162, P = 0.001; Fig. 4B). A positive correlation was obtained between log av-UCP mRNA level and log BMR (r = 0.426, P = 0.019; Fig. 4C), and a negative correlation was observed between log myostatin level and log BMR (r = −0.474, P = 0.008; Fig. 4D). A positive correlation was also obtained between log av-UCP mRNA level and log CCO activity level (r = 0.398, P = 0.029; Fig. 5B), and a negative correlation between log myostatin protein level and log CCO activity level (r = −0.391, P = 0.032; Fig. 5D).
Birds fasting for 12 h showed a significant decrease in serum glucose and triglyceride levels compared with the control birds, and the decreases were sustained for the 24-h duration of the experiment (Table 5). The fasting birds also had significantly lower serum T4 concentrations (Table 5). However, there was no significant difference in T3 concentration between the control group and each of the other fasting groups (Table 5). A positive correlation was obtained between log T3 and log BMR (r = 0.464, P = 0.010; Fig. 6). Positive correlations were also observed between log T3 and log state 4 respiration (r = 0.462, P = 0.010; Fig. 7A) and between log T3 and log CCO activity in the liver (r = 0.530, P = 0.003; Fig. 7B). Log T3 was positively associated with log av-UCP mRNA level (r = 0.339, P = 0.067; Fig. 7E) and negatively associated with log myostatin protein level (r = −0.333, P = 0.072; Fig. 7F), but the relationship did not quite attain significance.
Parameters | 0 h (n = 6) | 6 h (n = 6) | 12 h (n = 6) | 18 h (n = 6) | 24 h (n = 6) | F4,25 | P |
Glucose (mg dL−1) | 241.07 ± 4.75a | 183.55 ± 19.14ab | 161.04 ± 14.11bc | 157.34 ± 22.76bc | 96.40 ± 16.25c | 9.918 | <0.001 |
Triglyceride (mg dL−1) | 258.57 ± 36.37a | 210.48 ± 48.27ab | 98.62 ± 20.22bc | 65.69 ± 13.22c | 64.74 ± 7.25c | 9.303 | <0.001 |
T4 (ng mL−1) | 41.16 ± 6.65a | 40.01 ± 8.19ab | 32.37 ± 6.05ab | 23.64 ± 7.09ab | 14.04 ± 3.33b | 3.136 | 0.032 |
T3 (ng mL−1) | 3.42 ± 0.14ab | 3.61 ± 0.10a | 3.65 ± 0.10a | 3.44 ± 0.15ab | 3.04 ± 0.07b | 3.416 | 0.023 |
Note: statistical significance was determined by one-way ANOVA. Data are shown as mean ± SE from 30 birds. Different superscript letters in the same row indicate significant differences among groups after Tukey’s post hoc test. P-values in bold indicate differences with statistical significance. |
For endotherms, regulating thermogenetic indicators such as BMR, energy reserve, and aerobic capacity of cells in some visceral organs may be an effective way to adapt to unpredictable or reduced food resources (Karasov et al., 2004; McCue, 2010; Liknes and Swanson, 2011). In this study, fasting for 24 h resulted in lower body mass, BMR, and body fat in Eurasian Tree Sparrows, indicating that the energy expenditure of the birds was reduced. Furthermore, these birds also exhibited decreased state 4 respiration (in the liver), CCO activity (in both the liver and pectoral muscle), and levels of serum glucose, triglyceride, T4, and T3. In the muscle tissue, fasting also altered the expression of av-UCP and myostatin. Taken together, the data indicated that fasting reduced the oxidative substrates used in thermogenesis, resulting in lower energy consumption at a cellular level. The data also suggested that T3 may be involved in mediating thermogenesis in Tree Sparrows during fasting.
BMR is a highly variable trait, influenced by both genetics and environmental factors, including food resources (Cherel et al., 1994; Secor and Carey, 2016; Xu et al., 2024). To maintain energy balance during fasting, birds are likely to decrease their BMR in response to a lack of energy intake (Karasov et al., 2004; Xu et al., 2024). Several studies have demonstrated that a decrease in BMR can extend the duration for which stored energy reserves can sustain metabolism (Hiebert, 1991; Karasov et al., 2004; Xu et al., 2024). BMR was found to be significantly lower in the fasted Tree Sparrows, even if the fasting period was as short as 12 h (Fig. 1B). The phenotypic plasticity of BMR seen in Tree Sparrows may reflect their adaptability to food-restricted environments.
Body mass, which is used as a key indicator for the nutritional status of an animal, is maintained by balancing energy intake with expenditure (Kelly and Weathers, 2002; Hegemann et al., 2012). The lower body mass found in Tree Sparrows under food restriction (Fig. 1A) has been previously observed in other birds (Cherel and Le Maho, 1985; McCue, 2010; Secor and Carey, 2016). Zhao et al. (2014) claimed that body mass decrease is independent of age or sex but tends to depend on the diet, as well as the energy budget of the animals and the duration of food restriction. For example, reduced body mass was observed in White-throated Sparrows (Zonotrichia albicollis; Pierce and McWilliams, 2004), Chinese Bulbuls (Zhang et al., 2018a), and Silky Starlings (Mao et al., 2019), following a period of food restriction. Furthermore, decreased body mass may have contributed to the observed decrease in BMR, as indicated by the positive correlation between these two variables (Fig. 2A).
Body fat is another essential component that exhibits significant plasticity in response to environmental changes (Barzilai et al., 1998; Cooper, 2007; Zhang et al., 2024). During periods of food shortage, fat reserves can be used as emergency energy reserves (Zhang et al., 2018a; Mao et al., 2019). Many temperate passerines tend to accumulate body fat to buffer against temporary food shortages, and such a phenomenon has been observed for Dark-eyed Juncos (Junco hyemalis; Swanson, 1991), Juniper Titmice (Baeolophus ridgwayi; Cooper, 2007), and Mountain Chickadees (Poecile gambeli; Cooper, 2007). Consistent with the results of body mass and BMR, the birds fasted for 24 h demonstrated a significant loss in body fat compared with the control birds (Fig. 1D). Further, a positive correlation was also detected between body mass and body fat content (Fig. 2B), suggesting consistent changes for both body fat and body mass.
The mass or size of metabolically active organs, such as the liver, heart, kidney, and intestine, can influence the BMR of an animal (Daan et al., 1990; Williams and Tieleman, 2000; Zheng et al., 2008b, 2014a). Fasting Tree Sparrows showed a lighter liver, spleen, gizzard, and small intestine than the control sparrows (Table 1). These findings aligned with previous studies on small birds and suggested that changes in the masses of nutritional organs could be major contributors to BMR variation during fasting (Pierce and McWilliams, 2004; Zhang et al., 2018a; Xu et al., 2024). No significant decrease in pectoral muscle mass was detected for the fasting Tree Sparrows relative to the control ones. However, research has also demonstrated a positive correlation between BMR and skeletal muscle mass in various avian species, including House Sparrow (Passer domesticus; Chappell et al., 1999), Hwamei (Garrulax canorus; Zhou et al., 2016), and Tree Sparrow (Li et al., 2020), when the birds were provided with ad libitum access to food. Therefore, the differences in correlation between the pectoral muscle mass and BMR observed for different species may be related to whether the birds were subjected to fasting. Another possible reason is that birds may utilize the proteins released from the degradation of skeletal muscle to provide energy for the body at different time points during fasting. For example, silky starlings were found to have significantly decreased pectoral muscle mass accompanied by a significant decrease in BMR after 16 days of food restriction (Mao et al., 2019), indicating the initiation of protein degradation in skeletal muscle tissue to provide necessary metabolic substrates for the body. However, in the case of Tree Sparrows, no significant changes in pectoral muscle mass were detected after 24 h of fasting, and this was accompanied by a significant decrease in BMR, indicating these birds probably did not initiate protein degradation in the skeletal muscle tissue to provide metabolic substrates for the body, and therefore the status of the muscle mass did not correlate with BMR.
In endotherms, the liver ranks among the largest and most metabolically active organs, playing a significant role in the overall BMR (Zheng et al., 2014a; Zhou et al., 2016; Li et al., 2022). The skeletal muscle has a lower mass-specific metabolic rate, but because of the larger proportion of the total body mass, the skeletal muscle contributes significantly to thermogenesis in birds (Weber and Piersma, 1996; Chappell et al., 1999; Galt et al., 2014). The mechanisms of heat production in the liver and muscle include the uncoupling of oxidative phosphorylation, activities of key catabolic enzymes, and futile cycling of substrates (Swanson, 2010; Li et al., 2022, 2024). Many studies on birds have found positive correlations between BMR and state 4 respiration and/or CCO activity in various tissues under food restriction (Zhang et al., 2018a; Mao et al., 2019; Xu et al., 2024). For example, silky starlings subjected to 16 days of food restriction had significantly lower levels of state 4 respiration and CCO activity in the liver and pectoral muscle compared with the controls (Mao et al., 2019). Likewise, the same indicators in the same organ/tissue of Chinese bulbuls were also found to decrease significantly when subjected to fasting (Zhang et al., 2018a). In the present study, Tree Sparrows subjected to 24 h of fasting displayed a significant decrease in state 4 respiration in the liver relative to their control counterparts. In addition, these birds displayed lower levels of CCO activity in liver and pectoral muscle compared with the control birds. These results were consistent with those of previous studies (Mao et al., 2019; Xu et al., 2024). The low levels of state 4 respiration and CCO activity observed were related to decreased BMR, and such a finding was supported by the remarkable correlations between state 4 respiration and BMR and between CCO activity and BMR obtained for Tree Sparrows (Fig. 3A, B, D). Thus, modulation of the liver and pectoral muscle cellular thermogenesis may be one of the cellular mechanisms for decreasing BMR.
Blood glucose levels are the most commonly measured physiological variable for fasting animals (McCue, 2010). The impact of fasting on plasma glucose levels is different for birds. In some species, plasma glucose concentration remains constant or decreases at a certain point after fasting. For example, adult Greater Snow Geese (Anser caerulescens atlanticus) can maintain stable blood glucose levels during the second phase of fasting, but as they transition to the third phase, the blood glucose levels decrease (Boismenu et al., 1992). Female Japanese Quails (Xu et al., 2024) and emperor penguins (Groscolas, 1986) subjected to long-term fasting were found to have similar responses. Research has suggested that the physiological mechanism by which birds maintain glucose levels during fasting may be related to their unique ability to rapidly mobilize, distribute, and oxidize endogenous lipids (McCue, 2010). However, after fasting, the blood glucose levels of Tree Sparrows continued to decrease, suggesting that gluconeogenesis involving lipid mobilization may be insufficient to maintain blood glucose homeostasis during fasting.
Numerous investigations have indicated that glycogen serves as the principal energy source utilized by animals during fasting, especially in the initial phase (Rodríguez et al., 2005; Secor and Carey, 2016). For example, during the first 24 h of fasting, liver glycogen concentrations in bats and rodents were found to decrease by 85%–95% (Mosin, 1982; Freitas et al., 2003; Pinheiro et al., 2006; Mustonen et al., 2008). Similar to these mammals, the glycogen content in the liver of Tree Sparrows decreased by 70% after 24 h of fasting. King Penguins (Aptenodytes patagonicus) also use liver glycogen as an energy supply during the first phase fasting (Cherel and Le Maho, 1985). Glycogen from the skeletal muscle can also be depleted during fasting. For example, the muscle glycogen of Tree Sparrows significantly decreased after 18 h of fasting, and this increased glycogenolysis may temporarily offset the drain on circulating glucose levels (McCue, 2010).
The changes in plasma triglycerides vary among different avian species during fasting. For example, Herring Gulls (Larus argentatus) subjected to six days of fasting were found to have a 17% increase in plasma triglyceride level (Totzke et al., 1999). Similarly, the plasma triglyceride of the Yellow-legged Gull (Larus michahellis) was also shown to increase during the first 6 days of fasting and then decrease in the following 2 days (Alonso-Alvarez and Ferrer, 2001). Birds that show no significant changes in plasma triglyceride levels have also been reported, such as Japanese quails, in which the plasma triglyceride level displays no significant changes during prolonged fasting (Xu et al., 2024). On the contrary, the plasma triglycerides of Tree Sparrows significantly decreased after fasting for 12 h and reached the lowest level when the birds were fasted for 24 h. The products of triglyceride hydrolysis are fatty acids and glycerol, both of which can be converted to glucose through gluconeogenesis, so the serum triglyceride level is closely related to blood glucose level during fasting. Therefore, we speculate that the decrease in plasma triglyceride level may be an important reason for the decline in blood glucose level in Tree Sparrows during fasting, and such a decrease may be related to their limited fat reserves because of their small body size.
Uncoupling proteins (UCPs) are crucial for thermogenesis by dissipating the proton gradient across the mitochondrial membrane, thereby generating heat (Ricquier and Bouillaud, 2000; Davoodi et al., 2023). In birds, the av-UCP gene, which is a homolog of the UCP family, has been found to have a significant impact on various cellular processes, including thermogenesis of the skeletal muscle (Abe et al., 2006; Ferver and Dridi, 2020; Davoodi et al., 2023). Research has demonstrated that the expression levels of the av-UCP gene vary among different avian species' skeletal muscles when subjected to fasting conditions. For instance, Chinese Bulbuls exhibit a diminished expression of the av-UCP gene in their pectoral muscles after being fed only half of their regular food intake for a duration of 12 days (Zhang et al., 2018a). In a similar finding, king penguins also show a decrease in the av-UCP gene expression in their pectoral muscles following a 20-day fasting period (Rey et al., 2008). Recently, Japanese Quails have also been found to have significantly reduced expression of av-UCP in the pectoral muscles after 1–13 days of fasting (Xu et al., 2024). Similarly, the expression of the av-UCP gene in Tree Sparrows also decreased significantly during fasting as compared with no fasting. However, in quails, simultaneous decreases in S4R and CCO activity along with a decrease in av-UCP expression have also been observed in the pectoral muscle tissue (Xu et al., 2024). A decline in av-UCP gene expression during fasting may only contribute to a decrease in CCO activity in the pectoral muscle, as shown by the significant correlations found between CCO activity and av-UCP mRNA levels in our data. In summary, the decrease in av-UCP expression in the pectoral muscle in birds such as Tree Sparrows may be beneficial for the overall reduction of thermogenesis as it could result in decreased metabolic capacity of the pectoral muscle. Conversely, in chickens, fasting leads to a significant increase in av-UCP gene expression compared with no fasting (Toyomizu et al., 2006). Therefore, the relationship between av-UCP gene expression and regulation of thermogenesis by skeletal muscle during fasting requires additional investigation.
Myostatin functions as a muscle growth inhibitor, exerting negative regulation on muscle growth and development (McCroskery et al., 2003; Galt et al., 2014), and various studies have assessed myostatin expression levels in birds under fluctuating energy demands (Price et al., 2011; Zhang et al., 2018b). Recent studies have indicated that the expression of the myostatin gene tends to show an opposing trend with respect to thermogenic capacity in House Sparrows under seasonal acclimatization, with the expression level in summer exceeding that in winter by 1.52-fold (Swanson et al., 2009). Furthermore, cold and exercise have been shown to induce a rise in the metabolic capacity, consistent with a role for decreased myostatin expression to increase pectoral muscle mass in House Sparrows (Zhang et al., 2015b). Numerous studies have indicated that variation in myostatin expression among birds can lead to differences in muscle mass (Swanson et al., 2009, 2014; Price et al., 2011; King et al., 2015). However, the extent of support for the role of myostatin in regulating flexible muscle masses varies for different studies, with myostatin expression and muscle mass having a negative relationship (Swanson et al., 2009) and a positive or no relationship (Price et al., 2011). Our findings partially supported the notion that fasting leads to alterations in myostatin expression, although fasting in our case did not appear to affect the mass of the pectoral muscle (refer to Table 1). In Tree Sparrows, the expression of myostatin protein in the pectoral muscle was significantly higher during fasting (Fig. 4B). Moreover, the observed reduction in CCO activity during fasting (Table 3) implied that fluctuations in myostatin expression may play a role in the fasting-induced changes in muscle thermogenic capacity. The data collected from Tree Sparrows supported the hypothesis that elevated myostatin expression contributes to the lower metabolic capacity observed in fasting birds (Fig. 4D).
Thyroid hormones are crucial for regulating metabolic thermogenesis in endothermic organisms (Yen, 2001). Previous studies conducted by other investigators have established a significant correlation between thyroid hormones and obligatory thermogenesis (the heat generated from all the processes necessary for life, including digestion and assimilation of food, and corresponds to the BMR; Yen, 2001; Decuypere et al., 2005; Clapham, 2012; Tang et al., 2022). There are basically two major ways to generate more metabolic heat, namely, to increase ATP turnover and reduce the thermodynamic efficiency of ATP synthesis (Silva, 2006). Elevated serum levels of thyroid hormones have been demonstrated to enhance BMR across various avian species, such as Great Tits (Parus major; Silverin et al., 1989), Willow Tits (Poecile montanus; Silverin et al., 1989), Northern Cardinals (Cardinalis cardinalis; Burger and Denver, 2002), Little Buntings (Emberiza pusilla; Liu et al., 2006; Zheng et al., 2013), Tree Sparrows (Zheng et al., 2008b, 2014b), and Chinese Bulbuls (Zheng et al., 2014a; Tang et al., 2022). There is evidence to suggest that prolonged fasting in birds often leads to a decline in thyroid hormone levels and obligatory thermogenesis (Buyse et al., 2002; Rønning et al., 2009; Xu et al., 2024). The reduced serum T3 levels observed in fasting Tree Sparrows (Table 5) were consistent with the decrease in BMR. These results suggested that a decreased T3 level may play a role in the downregulation of BMR under fasting conditions, and this may be an important adaptation for Tree Sparrows to maintain energy balance when food resources are limited (Xu et al., 2024). In addition, our results also showed a significant decrease in T4 level but not the T3/T4 ratio (F4,25 = 2.352, P = 0.081). Past investigations have revealed a significant increase in T4 level but a decrease in the T3 to T4 ratio because of the less conversion of T4 to T3 (Edens et al., 1991; Van der Geyten et al., 1999). Moreover, T3 can affect mitochondrial proton leak and ATP turnover (Yen, 2001; Silva, 2006), in which T3 may uncouple oxidative phosphorylation in the mitochondria, resulting in changed state 4 respiration and CCO activity. An increase in thyroid hormone levels resulted in a corresponding rise in BMR, and this was associated with alterations in the activities of specific metabolic markers, including mitochondrial state 4 respiration and CCO across various tissues, and such observations have also been reported in previous studies (Liu et al., 2006; Zheng et al., 2008b, 2013, 2014a; Wen et al., 2019). The positive correlation that T3 level had with BMR (Fig. 6) as well as with state 4 respiration (Fig. 7A) and CCO activity in the liver (Fig. 7B) suggested that the activity of thyroid hormones might be vital to the variation in BMR during fasting. Moreover, thyroid hormones also influence substrate cycling (Yen, 2001; Silva, 2006; Mullur et al., 2014). Thyroid hormones are involved in the regulation of nutrient metabolism during fasting (Yen, 2001; Mullur et al., 2014). Thyroid hormones increase lipolysis and lipogenesis, glucose oxidation, and gluconeogenesis (Silva, 2006). The lower glucose and triglyceride levels in serum and glycogen levels in the liver and muscle displayed by fasting Tree Sparrows indicated a decrease in BMR, likely due to decreased thyroid hormone levels. In addition, thyroid hormones have the capacity to influence adaptive thermogenesis through the activation of uncoupling proteins (Yen, 2001; Silva, 2006; Mullur et al., 2014).
Thyroid hormones have been found to mediate muscle av-UCP-based heat production because T3 potentiates the transcription of the av-UCP gene (Collin et al., 2003). Furthermore, the synthesis of av-UCP mRNA has been demonstrated to be both induced and suppressed by elevated and reduced plasma T3 concentrations, respectively (Taouis et al., 2002; Collin et al., 2005; Xu et al., 2024). A positive correlation between T3 level and av-UCP mRNA level (Fig. 7E, P = 0.067, approached significance) suggested that thyroid hormones may participate in the thermoregulation of fasting sparrows by regulating the expression of av-UCP (Collin et al., 2003; Dridi et al., 2004). Thyroid hormones are also known to be involved in myostatin expression, but the results are variable (Ma et al., 2001; Carneiro et al., 2008). For example, rats administered T4 exhibited a significant increase in myostatin expression, both at the mRNA and protein levels (Ma et al., 2009). In contrast, Japanese quails subjected to long-term fasting were found to exhibit reduced expression of myostatin accompanied by decreased T3 level compared with the pre-fasting stage (Xu et al., 2024). Low levels of thyroid hormone are related to elevated levels of myostatin, a finding that was supported by the correlations between T3 and myostatin expression (Fig. 7F, P = 0.072, approached significance). Thus, in Tree Sparrows that underwent fasting, myostatin expression might be regulated by thyroid hormones and influenced metabolic thermogenesis.
Eurasian Tree Sparrows subjected to food restriction displayed a number of changes indicative of alteration in thermogenesis and metabolism. These changes included variations in body mass, BMR, body fat, internal organ masses, cellular metabolic indicators in the liver and muscle, av-UCP and myostatin expression in the pectoral muscle, substrate cycling, and serum T3 levels. These findings could suggest a decrease in thermogenic capacity as an adaptive response to food shortages. The observed correlations between BMR and markers of aerobic metabolism in the liver and muscle indicated that the reductions in state 4 respiration and CCO activity found in this study were likely to be related to BMR variation. Moreover, av-UCP and myostatin in the pectoral muscle may potentially be involved in metabolic thermogenesis. The data also suggested the involvement of T3 in mediating the regulation of thermogenesis in sparrows. Collectively, these data illustrated the involvement of morphological, physiological, enzymatic, and endocrine adjustments that facilitate high phenotypic flexibility in basal thermogenesis in Tree Sparrows during fasting.
Hong Jin: Writing – original draft, Data curation. Qi Xia: Writing – original draft, Methodology. Ziqing Gao: Software, Formal analysis. Yujie XuanYuan: Software, Formal analysis. Beibei Dong: Formal analysis. Ming Li: Writing – review & editing, Funding acquisition. Qingjian Liang: Writing – original draft, Methodology, Conceptualization. Jinsong Liu: Writing – review & editing, Project administration, Funding acquisition.
All experimental protocols received approval from the Animal Care and Use Committee of Wenzhou University (No. WZU-045).
The authors declare that they have no known competing financial interests or personal relationships that could have appeared to influence the work reported in this paper.
We express our gratitude to Dr. Alan K. Chang from the College of Life and Environmental Sciences at Wenzhou University for his valuable assistance in refining the language of this manuscript. We also thank the anonymous reviewers for their helpful comments and suggestions.
Supplementary data to this article can be found online at https://doi.org/10.1016/j.avrs.2024.100220.
Boismenu, C., Gauthier, G., Larochelle, J., 1992. Physiology of prolonged fasting in greater snow geese (Chen caerulescens atlantica). Auk 109, 511–521.
|
Cherel, Y., Le Maho, Y., 1985. Five months of fasting in king penguin chicks: body mass loss and fuel metabolism. Am. J. Physiol. 249, R387–R392.
|
Daan, S., Masman, D., Groenewold, A., 1990. Avian basal metabolic rates: their association with body composition and energy expenditure in nature. Am. J. Physiol. 259, R333–R340.
|
Hohtola, E., 2012. Thermoregulatory adaptations to starvation in birds. In: McCue, M.D. (Ed.), Comparative Physiology of Fasting, Starvation, and Food Limitation. Springer-Verlag, San Antonio, TX, USA, pp. 157–170.
|
Hu, S.N., Zhu, Y.Y., Lin, L., Zheng, W.H., Liu, J.S., 2017. Temperature and photoperiod as environmental cues affect body mass and thermoregulation in Chinese bulbuls, Pycnonotus sinensis. J. Exp. Biol. 220, 844–855.
|
Liang, Q.J., Zhao, L., Wang, J.Q., Chen, Q., Zheng, W.H., Liu, J.S., 2015. Effect of food restriction on the energy metabolism of the Chinese bulbul (Pycnonotus sinensis). Zool. Res. 36, 79–87.
|
Liu, J.S., Li, M., 2006. Phenotypic flexibility of metabolic rate and organ masses among tree sparrows Passer montanus in seasonal acclimatization. Acta Zool. Sin. 52, 469–477.
|
MacKinnon, J., Phillipps, K., 2000. A Field Guide to the Birds of China. Oxford University Press, London, pp. 491–493..
|
McCue, M.D., 2010. Starvation physiology: reviewing the different strategies animals use to survive a common challenge. Comp. Biochem. Physiol. A 156, 1–18.
|
Pinheiro, E.C., Taddei, V.A., Migliorini, R.H., Kettelhut, I.C., 2006. Effect of fasting on carbohydrate metabolism in frugivorous bats (Artibeus lituratus and Artibeus jamaicensis). Comp. Biochem. Physiol. B 143, 279–284.
|
Schmidt-Nielsen, K., 1997. Animal Physiology: Adaptation and Environment. Cambridge University Press, Cambridge..
|
Swanson, D.L., 2010. Seasonal metabolic variation in birds: functional and mechanistic correlates. In: Thompson, C.F. (Ed.), Current Ornithology, vol. 17. Springer, Berlin, pp. 75–129.
|
Zhang, Y.F., Eyster, K., Liu, J.S., Swanson, D.L., 2015. Cross-training in birds: cold and exercise training produce similar changes in maximal metabolic output, muscle masses and myostatin expression in house sparrows (Passer domesticus). J. Exp. Biol. 218, 2190–2200.
|
Zhao, Z.J., Liu, Y.A., Xing, J.Y., Zhang, M.L., Ni, X.Y., Cao, J., 2014. The role of leptin in striped hamsters subjected to food restriction and refeeding. Zool. Res. 35, 262–271.
|
Zheng, W.H., Li, M., Liu, J.S., Shao, S.L., 2008b. Seasonal acclimatization of metabolism in Eurasian tree sparrows (Passer montanus). Comp. Biochem. Physiol. A 151, 519–525.
|
Zheng, W.H., Lin, L., Liu, J.S., Xu, X.J., Li, M., 2013. Geographic variation in basal thermogenesis in little buntings: relationship to cellular thermogenesis and thyroid hormone concentrations. Comp. Biochem. Physiol. A 164, 240–246.
|
Parameters | 0 h (n = 6) | 6 h (n = 6) | 12 h (n = 6) | 18 h (n = 6) | 24 h (n = 6) | P | |
Wet mass (g) | |||||||
Pectoral muscle | 1.76 ± 0.13 | 1.89 ± 0.12 | 2.06 ± 0.12 | 1.89 ± 0.12 | 2.05 ± 0.13 | χ2 = 2.933 | 0.569 |
Liver | 0.79 ± 0.07 a | 0.80 ± 0.06 a | 0.79 ± 0.06 a | 0.65 ± 0.06 ab | 0.49 ± 0.07 b | F4,24 = 3.650 | 0.018 |
Heart | 0.19 ± 0.01ab | 0.20 ± 0.01a | 0.20 ± 0.01a | 0.17 ± 0.01b | 0.17 ± 0.01b | F4,24 = 2.870 | 0.045 |
Kidney | 0.16 ± 0.02 | 0.18 ± 0.01 | 0.19 ± 0.01 | 0.19 ± 0.01 | 0.16 ± 0.02 | F4,24 = 1.065 | 0.395 |
Lung | 0.16 ± 0.01b | 0.20 ± 0.01a | 0.17 ± 0.01b | 0.16 ± 0.01b | 0.15 ± 0.01b | F4,24 = 3.950 | 0.013 |
Spleen | 0.03 ± 0.00 a | 0.03 ± 0.00 a | 0.02 ± 0.00 ab | 0.02 ± 0.00 bc | 0.01 ± 0.00 c | F4,24 = 4.316 | 0.009 |
Brain | 0.77 ± 0.03a | 0.81 ± 0.03a | 0.69 ± 0.03b | 0.77 ± 0.03a | 0.77 ± 0.03a | F4,24 = 3.173 | 0.032 |
Gizzard | 0.52 ± 0.03ab | 0.47 ± 0.03abc | 0.54 ± 0.03a | 0.46 ± 0.03bc | 0.41 ± 0.03c | F4,24 = 3.186 | 0.031 |
Small intestine | 0.54 ± 0.05a | 0.55 ± 0.04a | 0.51 ± 0.04a | 0.42 ± 0.04b | 0.35 ± 0.05b | F4,24 = 3.061 | 0.036 |
Total digestive tract | 1.10 ± 0.06a | 1.08 ± 0.05a | 1.11 ± 0.05a | 0.91 ± 0.05b | 0.80 ± 0.06b | F4,24 = 5.686 | 0.002 |
Note: Statistical significance was determined by one-way ANCOVA except for pectoral muscle. For pectoral muscle, statistical significance was determined by Kruskal–Wallis. Data are shown as mean ± SE from 30 birds. Different superscript letters in the same row indicate significant differences among groups after Tukey’s post hoc test. P-values in bold indicate differences with statistical significance. |
Allometric correlationsa | Pectoral muscle | Liver | Heart | Kidney | Lung | Spleen | Brain | Gizzard | Small intestine | Total digestive tract | |
r | 0.561 | 0.385 | 0.642 | 0.182 | 0.231 | 0.445 | 0.254 | 0.171 | 0.468 | 0.447 | |
P | 0.001 | 0.035 | <0.001 | 0.337 | 0.221 | 0.014 | 0.175 | 0.366 | 0.009 | 0.013 | |
Slope | 1.012 | 0.979 | 0.855 | 0.325 | 0.333 | 1.988 | 0.223 | 0.237 | 1.389 | 0.767 | |
Residual correlationsb | |||||||||||
r | 0.146 | 0.492 | 0.351 | 0.114 | 0.398 | 0.423 | 0.237 | 0.247 | 0.353 | 0.379 | |
P | 0.442 | 0.006 | 0.057 | 0.550 | 0.029 | 0.020 | 0.208 | 0.188 | 0.056 | 0.039 | |
Slope | −0.094 | 0.202 | 0.332 | 0.091 | 0.398 | 0.102 | 0.389 | 0.258 | 0.130 | 0.238 | |
a Linear regression statistics for log organ mass versus log body mass (minus wet mass of the organ) allometric equations (allometric correlations). b Linear regression statistics for log organ mass residuals versus log BMR residuals (residual correlations), or log organ mass versus log BMR raw values if allometric equations were not significant. P-values in bold indicate differences with statistical significance. |
Variable | Control (n = 6) | 6 h (n = 6) | 12 h (n = 6) | 18 h (n = 6) | 24 h (n = 6) | F4,25 | P |
Liver: | |||||||
State 4 respiration (μmol O2 min−1 organ−1) | 0.27 ± 0.03a | 0.27 ± 0.01a | 0.26 ± 0.03a | 0.25 ± 0.02ab | 0.18 ± 0.01b | 2.846 | 0.045 |
Cytochrome c oxidase activity (μmol O2 min−1 organ−1) | 0.12 ± 0.01a | 0.12 ± 0.01ab | 0.11 ± 0.01ab | 0.10 ± 0.00ab | 0.09 ± 0.01b | 3.966 | 0.013 |
Muscle: | |||||||
State 4 respiration (μmol O2 min−1 whole tissue−1) | 1.01 ± 0.13 | 1.05 ± 0.18 | 0.98 ± 0.08 | 0.91 ± 0.10 | 0.88 ± 0.05 | 0.379 | 0.822 |
Cytochrome c oxidase activity (μmol O2 min−1 whole tissue−1) | 0.49 ± 0.06a | 0.44 ± 0.02a | 0.45 ± 0.04a | 0.41 ± 0.03ab | 0.29 ± 0.03b | 4.398 | 0.008 |
Note: statistical significance was determined by one-way ANOVA. Data are shown as mean ± SE from 30 birds. Different superscript letters in the same row indicate significant differences among groups after Tukey’s post hoc test. P-values in bold indicate differences with statistical significance. |
Parameters | 0 h (n = 6) | 6 h (n = 6) | 12 h (n = 6) | 18 h (n = 6) | 24 h (n = 6) | P | |
Liver glycogen (mg g−1) | 2.66 ± 0.15a | 1.77 ± 0.22a | 1.12 ± 0.07ab | 0.81 ± 0.06b | 0.79 ± 0.04b | χ2 = 25.144 | <0.001 |
Muscle glycogen (mg g−1) | 1.22 ± 0.16a | 0.89 ± 0.07 ab | 0.84 ± 0.08 abc | 0.65 ± 0.09bc | 0.45 ± 0.07c | F4,25 = 7.877 | <0.001 |
Note: For muscle glycogen, statistical significance was determined by one-way ANOVA; for liver glycogen, statistical significance was determined by Kruskal-Wallis. Data are shown as mean ± SE from 30 birds. Different superscript letters in the same row indicate significant differences among groups after Tukey’s post hoc test. P-values in bold indicate differences with statistical significance. |
Parameters | 0 h (n = 6) | 6 h (n = 6) | 12 h (n = 6) | 18 h (n = 6) | 24 h (n = 6) | F4,25 | P |
Glucose (mg dL−1) | 241.07 ± 4.75a | 183.55 ± 19.14ab | 161.04 ± 14.11bc | 157.34 ± 22.76bc | 96.40 ± 16.25c | 9.918 | <0.001 |
Triglyceride (mg dL−1) | 258.57 ± 36.37a | 210.48 ± 48.27ab | 98.62 ± 20.22bc | 65.69 ± 13.22c | 64.74 ± 7.25c | 9.303 | <0.001 |
T4 (ng mL−1) | 41.16 ± 6.65a | 40.01 ± 8.19ab | 32.37 ± 6.05ab | 23.64 ± 7.09ab | 14.04 ± 3.33b | 3.136 | 0.032 |
T3 (ng mL−1) | 3.42 ± 0.14ab | 3.61 ± 0.10a | 3.65 ± 0.10a | 3.44 ± 0.15ab | 3.04 ± 0.07b | 3.416 | 0.023 |
Note: statistical significance was determined by one-way ANOVA. Data are shown as mean ± SE from 30 birds. Different superscript letters in the same row indicate significant differences among groups after Tukey’s post hoc test. P-values in bold indicate differences with statistical significance. |